There are sometimes individuals who seem always to find themselves at the focal points of their times. The physicist George Uhlenbeck was one of these individuals, showing up at all the right times in all the right places at the dawn of modern physics in the 1920’s and 1930’s. He studied under Ehrenfest and Bohr and Born, and he was friends with Fermi and Oppenheimer and Oskar Klein. He taught physics at the universities at Leiden, Michigan, Utrecht, Columbia, MIT and Rockefeller. He was a wide-ranging theoretical physicist who worked on Brownian motion, early string theory, quantum tunneling, and the master equation. Yet he is most famous for the very first thing he did as a graduate student—the discovery of the quantum spin of the electron.
Electron Spin
G. E. Uhlenbeck, and S. Goudsmit, “Spinning electrons and the structure of spectra,” Nature 117, 264-265 (1926).
George Uhlenbeck (1900 – 1988) was born in the Dutch East Indies, the son of a family with a long history in the Dutch military [1]. After the father retired to The Hague, George was expected to follow the family tradition into the military, but he stumbled onto a copy of H. Lorentz’ introductory physics textbook and was hooked. Unfortunately, to attend university in the Netherlands at that time required knowledge of Greek and Latin, which he lacked, so he entered the Institute of Technology in Delft to study chemical engineering. He found the courses dreary.
Fortunately, he was only a few months into his first semester when the language requirement was dropped, and he immediately transferred to the University of Leiden to study physics. He tried to read Boltzmann, but found him opaque, but then read the famous encyclopedia article by the husband and wife team of Paul and Tatiana Ehrenfest on statistical mechanics (see my Physics Today article [2]), which became his lifelong focus.
After graduating, he continued into graduate school, taking classes from Ehrenfest, but lacking funds, he supported himself by teaching classes at a girls high school, until he heard of a job tutoring the son of the Dutch ambassador to Italy. He was off to Rome for three years, where he met Enrico Fermi and took classes from Tullio Bevi-Cevita and Vito Volterra.
However, he nearly lost his way. Surrounded by the rich cultural treasures of Rome, he became deeply interested in art and was seriously considering giving up physics and pursuing a degree in art history. When Ehrenfest got wind of this change in heart, he recalled Uhlenbeck in 1925 to the Netherlands and shrewdly paired him up with another graduate student, Samuel Goudsmit, to work on a new idea proposed by Wolfgang Pauli a few months earlier on the exclusion principle.
Pauli had explained the filling of the energy levels of atoms by introducing a new quantum number that had two values. Once an energy level was filled by two electrons, each carrying one of the two quantum numbers, this energy level “excluded” any further filling by other electrons.
To Uhlenbeck, these two quantum numbers seemed as if they must arise from some internal degree of freedom, and in a flash of insight he imagined that it might be caused if the electron were spinning. Since spin was a form of angular momentum, the spin degree of freedom would combine with orbital angular momentum to produce a composite angular momentum for the quantum levels of atoms.
The idea of electron spin was not immediately embraced by the broader community, and Bohr and Heisenberg and Pauli had their reservations. Fortunately, they all were traveling together to attend the 50th anniversary of Lorentz’ doctoral examination and were met at the train station in Leiden by Ehrenfest and Einstein. As usual, Einstein had grasped the essence of the new physics and explained how the moving electron feels an induced magnetic field which would act on the magnetic moment of the electron to produce spin-orbit coupling. With that, Bohr was convinced.
Uhlenbeck and Goudsmit wrote up their theory in a short article in Nature, followed by a short note by Bohr. A few months later, L. H. Thomas, while visiting Bohr in Copenhagen, explained the factor of two that appears in (what later came to be called) Thomas precession of the electron, cementing the theory of electron spin in the new quantum mechanics.
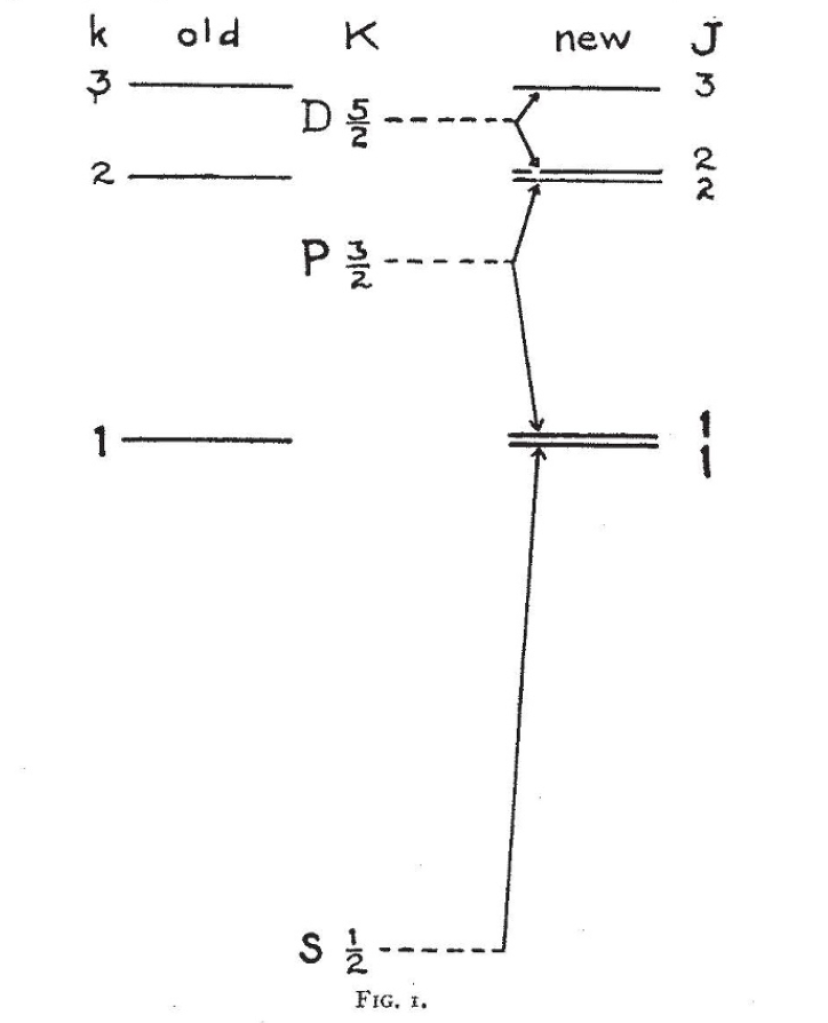
5-Dimensional Quantum Mechanics
P. Ehrenfest, and G. E. Uhlenbeck, “Graphical illustration of De Broglie’s phase waves in the five-dimensional world of O Klein,” Zeitschrift Fur Physik 39, 495-498 (1926).
Around this time, the Swedish physicist Oskar Klein visited Leiden after returning from three years at the University of Michigan where he had taken advantage of the isolation to develop a quantum theory of 5-dimensional spacetime. This was one of the first steps towards a grand unification of the forces of nature since there was initial hope that gravity and electromagnetism might both be expressed in terms of the five-dimensional space.
An unusual feature of Klein’s 5-dimensional relativity theory was the compactness of the fifth dimension, in which it was “rolled up” into a kind of high-dimensional string with a tiny radius. If the 4-dimensional theory of spacetime was sometimes hard to visualize, here was an even tougher problem.
Uhlenbeck and Ehrenfest met often with Klein during his stay in Leiden, discussing the geometry and consequences of the 5-dimensional theory. Ehrenfest was always trying to get at the essence of physical phenomena in the simplest terms. His famous refrain was “Was ist der Witz?” (What is the point?) [1]. These discussions led to a simple paper in Zeitschrift für Physik published later that year in 1926 by Ehrenfest and Uhlenbeck with the compelling title “Graphical Illustration of De Broglie’s Phase Waves in the Five-Dimensional World of O Klein”. The paper provided the first visualization of the 5-dimensional spacetime with the compact dimension. The string-like character of the spacetime was one of the first forays into modern day “string theory” whose dimensions have now expanded to 11 from 5.
During his visit, Klein also told Uhlenbeck about the relativistic Schrödinger equation that he was working on, which would later become the Klein-Gordon equation. This was a near miss, because what the Klein-Gordon equation was missing was electron spin—which Uhlenbeck himself had introduced into quantum theory—but it would take a few more years before Dirac showed how to incorporate spin into the theory.
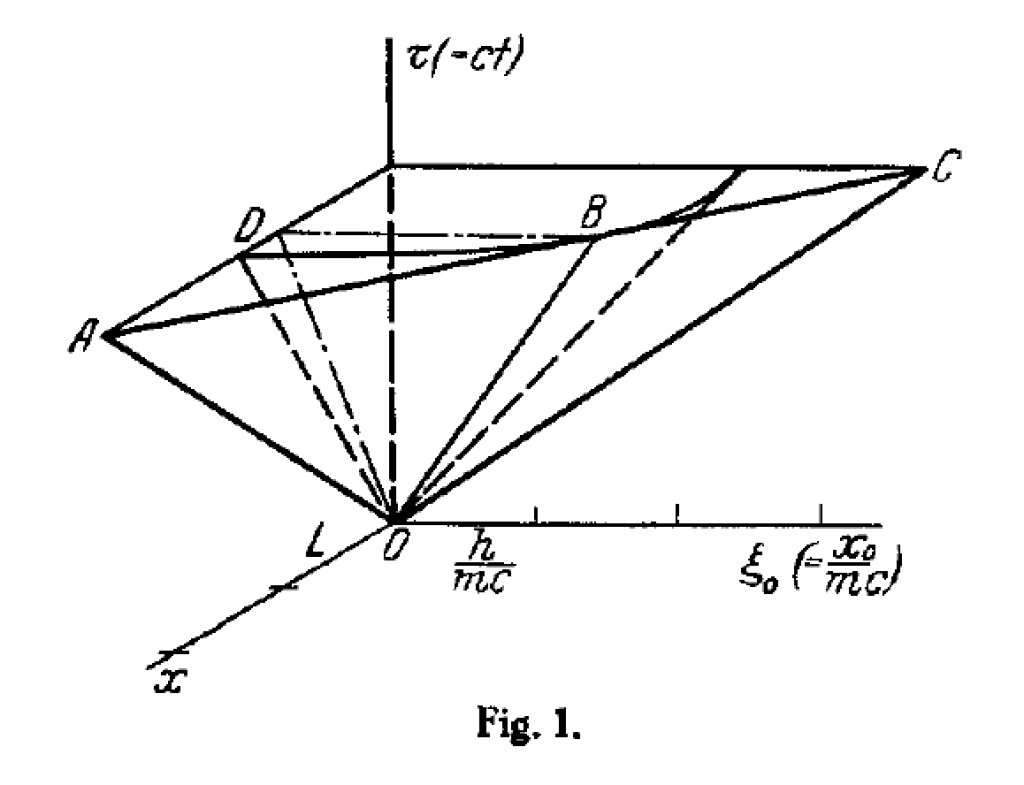
Brownian Motion
G. E. Uhlenbeck and L. S. Ornstein, “On the theory of the Brownian motion,” Physical Review 36, 0823-0841 (1930).
After spending time with Bohr in Copenhagen while finishing his PhD, Uhlenbeck visited Max Born at Göttingen where he met J. Robert Oppenheimer who was also visiting Born at that time. When Uhlenbeck traveled to the United States in late summer of 1927 to take a position at the University of Michigan, he was met at the dock in New York by Oppenheimer.
Uhlenbeck was a professor of physics at Michigan for eight years from 1927 to 1935, and he instituted a series of Summer Schools [3] in theoretical physics that attracted international participants and introduced a new generation of American physicists to the rigors of theory that they previously had to go to Europe to find.
In this way, Uhlenbeck was part of a great shift that occurred in the teaching of graduate-level physics of the 1930’s that brought European expertise to the United States. Just a decade earlier, Oppenheimer had to go to Göttingen to find the kind of education that he needed for graduate studies in physics. Oppenheimer brought the new methods back with him to Berkeley, where he established a strong theory department to match the strong experimental activities of E. O. Lawrence. Now, European physicists too were coming to America, an exodus accelerated by the increasing anti-Semitism in Europe under the rise of fascism.
During this time, one of Uhlenbeck’s collaborators was L. S. Ornstein, the director of the Physical Laboratory at the University of Utrecht and a founding member of the Dutch Physical Society. Uhlenbeck and Ornstein were both interested in the physics of Brownian motion, but wished to establish the phenomenon on a more sound physical basis. Einstein’s famous paper of 1905 on Brownian motion had made several Einstein-style simplifications that stripped the complicated theory to its bare essentials, but had lost some of the details in the process, such as the role of inertia at the microscale.
Uhlenbeck and Ornstein published a paper in 1930 that developed the stochastic theory of Brownian motion, including the effects of particle inertia. The stochastic differential equation (SDE) for velocity is

where γ is viscosity, Γ is a fluctuation coefficient, and dw is a “Wiener process”. The Wiener differential dw has unusual properties such that
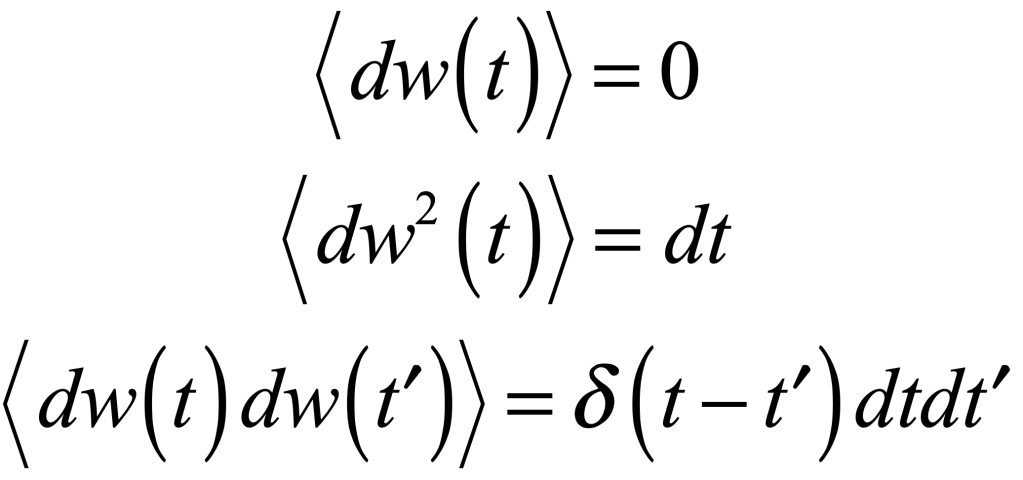
Uhlenbeck and Ornstein solived this SDE to yield an average velocity
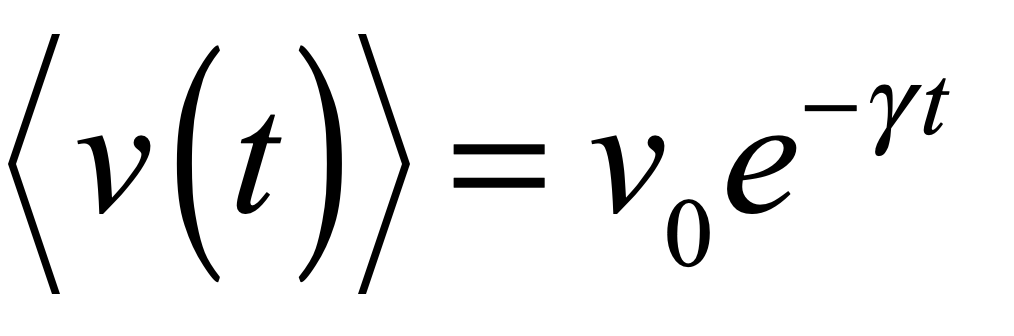
which decays to zero at long times, and a variance
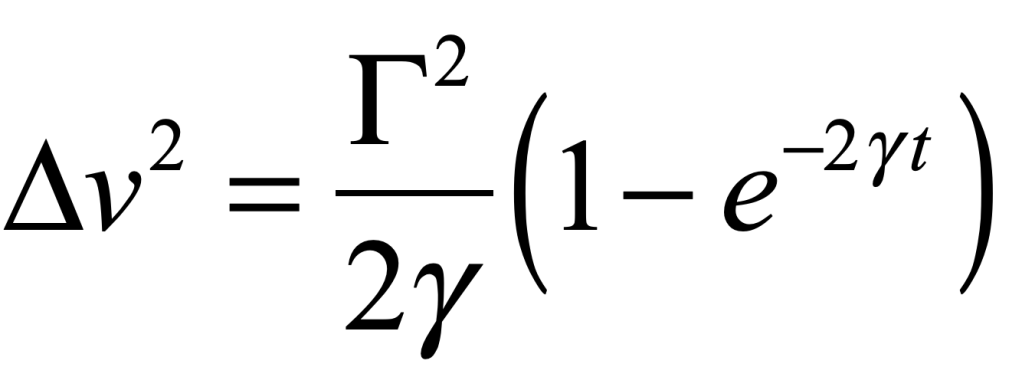
that asymptotes to a finite value at long times. The fluctuation coefficient is thus given by
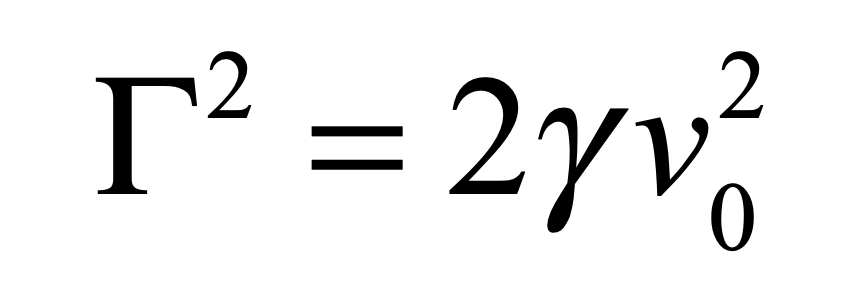
for a process with characteristic speed v0. An estimate for the fluctuation coefficient can be obtained by considering the force F on an object of size a
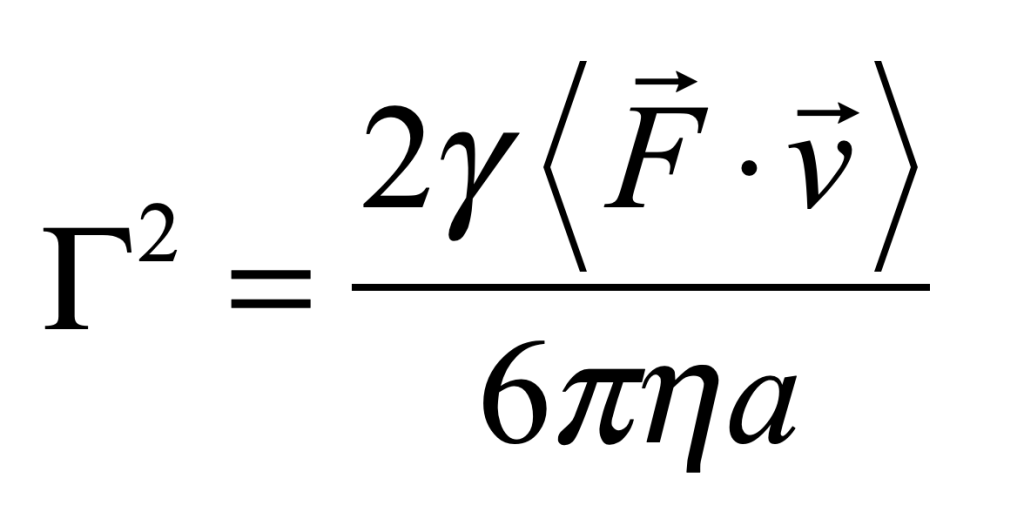
For instance, for intracellular transport [4], the fluctuation coefficient has a rough value of Γ = 2 Hz μm2/sec2.
Quantum Tunneling
D. M. Dennison and G. E. Uhlenbeck, “The two-minima problem and the ammonia molecule,” Physical Review 41, 313-321 (1932).
By the early 1930’s, quantum tunnelling of the electron through classically forbidden regions of potential energy was well established, but electrons did not have a monopoly on quantum effects. Entire atoms—electrons plus nucleus—also have quantum wave functions and can experience regions of classically forbidden potential.
Uhlenbeck, with David Dennison, a fellow physicist at Ann Arbor, Michigan, developed the first quantum theory of molecular tunneling for the molecular configuration of ammonia NH3 that can tunnel between the two equivalent configurations. Their use of the WKB approximation in the paper set the standard for subsequent WKB approaches that would play an important role in the calculation of nuclear decay rates.

Master Equation
A. Nordsieck, W. E. Lamb, and G. E. Uhlenbeck, “On the theory of cosmic-ray showers I. The furry model and the fluctuation problem,” Physica 7, 344-360 (1940)
In 1935, Uhlenbeck left Michigan to take up the physics chair recently vacated by Kramers at Utrecht. However, watching the rising Nazism in Europe, he decided to return to the United States, beginning as a visiting professor at Columbia University in New York in 1940. During his visit, he worked with W. E. Lamb and A. Nordsieck on the problem of cosmic ray showers.
Their publication on the topic included a rate equation that is encountered in a wide range of physical phenomena. They called it the “Master Equation” for ease of reference in later parts of the paper, but this phrase stuck, and the “Master Equation” is now a standard tool used by physicists when considering the balances among multiples transitions.
Uhlenbeck never returned to Europe, moving among Michigan, MIT, Princeton and finally settling at Rockefeller University in New York from where he retired in 1971.
Selected Works by George Uhlenbeck:
G. E. Uhlenbeck, and S. Goudsmit, “Spinning electrons and the structure of spectra,” Nature 117, 264-265 (1926).
P. Ehrenfest, and G. E. Uhlenbeck, “On the connection of different methods of solution of the wave equation in multi dimensional spaces,” Proceedings of the Koninklijke Akademie Van Wetenschappen Te Amsterdam 29, 1280-1285 (1926).
P. Ehrenfest, and G. E. Uhlenbeck, “Graphical illustration of De Broglie’s phase waves in the five-dimensional world of O Klein,” Zeitschrift Fur Physik 39, 495-498 (1926).
G. E. Uhlenbeck, and L. S. Ornstein, “On the theory of the Brownian motion,” Physical Review 36, 0823-0841 (1930).
D. M. Dennison, and G. E. Uhlenbeck, “The two-minima problem and the ammonia molecule,” Physical Review 41, 313-321 (1932).
E. Fermi, and G. E. Uhlenbeck, “On the recombination of electrons and positrons,” Physical Review 44, 0510-0511 (1933).
A. Nordsieck, W. E. Lamb, and G. E. Uhlenbeck, “On the theory of cosmic-ray showers I The furry model and the fluctuation problem,” Physica 7, 344-360 (1940).
M. C. Wang, and G. E. Uhlenbeck, “On the Theory of the Brownian Motion-II,” Reviews of Modern Physics 17, 323-342 (1945).
G. E. Uhlenbeck, “50 Years of Spin – Personal Reminiscences,” Physics Today 29, 43-48 (1976).
Notes:
[1] George Eugene Uhlenbeck: A Biographical Memoire by George Ford (National Academy of Sciences, 2009). https://www.nasonline.org/publications/biographical-memoirs/memoir-pdfs/uhlenbeck-george.pdf
[2] D. D. Nolte, “The tangled tale of phase space,” Physics Today 63, 33-38 (2010).
[3] One of these was the famous 1948 Summer School session where Freeman Dyson met Julian Schwinger after spending days on a cross-country road trip with Richard Feynman. Schwinger and Feynman had developed two different approaches to quantum electrodynamics (QED), which Dyson subsequently reconciled when he took up his position later that year at Princeton’s Institute for Advanced Study, helping to launch the wave of QED that spread out over the theoretical physics community.
[4] D. D. Nolte, “Coherent light scattering from cellular dynamics in living tissues,” Reports on Progress in Physics 87 (2024).