At the dawn of quantum theory, Heisenberg, Schrödinger, Bohr and Pauli were embroiled in a dispute over whether trajectories of particles, defined by their positions over time, could exist. The argument against trajectories was based on an apparent paradox: To draw a “line” depicting a trajectory of a particle along a path implies that there is a momentum vector that carries the particle along that path. But a line is a one-dimensional curve through space, and since at any point in time the particle’s position is perfectly localized, then by Heisenberg’s uncertainty principle, it can have no definable momentum to carry it along.
My previous blog shows the way out of this paradox, by assembling wavepackets that are spread in both space and momentum, explicitly obeying the uncertainty principle. This is nothing new to anyone who has taken a quantum course. But the surprising thing is that in some potentials, like a harmonic potential, the wavepacket travels without broadening, just like classical particles on a trajectory. A dramatic demonstration of this can be seen in this YouTube video. But other potentials “break up” the wavepacket, especially potentials that display classical chaos. Because phase space is one of the best tools for studying classical chaos, especially Hamiltonian chaos, it can be enlisted to dig deeper into the question of the quantum trajectory—not just about the existence of a quantum trajectory, but why quantum systems retain a shadow of their classical counterparts.
Phase Space
Phase space is the state space of Hamiltonian systems. Concepts of phase space were first developed by Boltzmann as he worked on the problem of statistical mechanics. Phase space was later codified by Gibbs for statistical mechanics and by Poincare for orbital mechanics, and it was finally given its name by Paul and Tatiana Ehrenfest (a husband-wife team) in correspondence with the German physicist Paul Hertz (See Chapter 6, “The Tangled Tale of Phase Space”, in Galileo Unbound by D. D. Nolte (Oxford, 2018)).
The stretched-out phase-space functions … are very similar to the stochastic layer that forms in separatrix chaos in classical systems.
The idea of phase space is very simple for classical systems: it is just a plot of the momentum of a particle as a function of its position. For a given initial condition, the trajectory of a particle through its natural configuration space (for instance our 3D world) is traced out as a path through phase space. Because there is one momentum variable per degree of freedom, then the dimensionality of phase space for a particle in 3D is 6D, which is difficult to visualize. But for a one-dimensional dynamical system, like a simple harmonic oscillator (SHO) oscillating in a line, the phase space is just two-dimensional, which is easy to see. The phase-space trajectories of an SHO are simply ellipses, and if the momentum axis is scaled appropriately, the trajectories are circles. The particle trajectory in phase space can be animated just like a trajectory through configuration space as the position and momentum change in time p(x(t)). For the SHO, the point follows the path of a circle going clockwise.

A more interesting phase space is for the simple pendulum, shown in Fig. 2. There are two types of orbits: open and closed. The closed orbits near the origin are like those of a SHO. The open orbits are when the pendulum is spinning around. The dividing line between the open and closed orbits is called a separatrix. Where the separatrix intersects itself is a saddle point. This saddle point is the most important part of the phase space portrait: it is where chaos emerges when perturbations are added.

One route to classical chaos is through what is known as “separatrix chaos”. It is easy to see why saddle points (also known as hyperbolic points) are the source of chaos: as the system trajectory approaches the saddle, it has two options of which directions to go. Any additional degree of freedom in the system (like a harmonic drive) can make the system go one way on one approach, and the other way on another approach, mixing up the trajectories. An example of the stochastic layer of separatrix chaos is shown in Fig. 3 for a damped driven pendulum. The chaotic behavior that originates at the saddle point extends out along the entire separatrix.

The main question about whether or not there is a quantum trajectory depends on how quantum packets behave as they approach a saddle point in phase space. Since packets are spread out, it would be reasonable to assume that parts of the packet will go one way, and parts of the packet will go another. But first, one has to ask: Is a phase-space description of quantum systems even possible?
Quantum Phase Space: The Wigner Distribution Function
Phase-space portraits are arguably the most powerful tool in the toolbox of classical dynamics, and one would like to retain its uses for quantum systems. However, there is that pesky paradox about quantum trajectories that cannot admit the existence of one-dimensional curves through such a phase space. Furthermore, there is no direct way of taking a wavefunction and simply “finding” its position or momentum to plot points on such a quantum phase space.
The answer was found in 1932 by Eugene Wigner (1902 – 1905), an Hungarian physicist working at Princeton. He realized that it was impossible to construct a quantum probability distribution in phase space that had positive values everywhere. This is a problem, because negative probabilities have no direct interpretation. But Wigner showed that if one relaxed the requirements a bit, so that expectation values computed over some distribution function (that had positive and negative values) gave correct answers that matched experiments, then this distribution function would “stand in” for an actual probability distribution.
The distribution function that Wigner found is called the Wigner distribution function. Given a wavefunction ψ(x), the Wigner distribution is defined as

The Wigner distribution function is the Fourier transform of the convolution of the wavefunction. The pure position dependence of the wavefunction is converted into a spread-out position-momentum function in phase space. For a Gaussian wavefunction ψ(x) with a finite width in space, the W-function in phase space is a two-dimensional Gaussian with finite widths in both space and momentum. In fact, the Δx-Δp product of the W-function is precisely the uncertainty production of the Heisenberg uncertainty relation.
The question of the quantum trajectory from the phase-space perspective becomes whether a Wigner function behaves like a localized “packet” that evolves in phase space in a way analogous to a classical particle, and whether classical chaos is reflected in the behavior of quantum systems.
The Harmonic Oscillator
The quantum harmonic oscillator is a rare and special case among quantum potentials, because the energy spacings between all successive states are all the same. This makes it possible for a Gaussian wavefunction, which is a superposition of the eigenstates of the harmonic oscillator, to propagate through the potential without broadening. To see an example of this, watch the first example in this YouTube video for a Schrödinger cat state in a two-dimensional harmonic potential. For this very special potential, the Wigner distribution behaves just like a (broadened) particle on an orbit in phase space, executing nice circular orbits.
A comparison of the classical phase-space portrait versus the quantum phase-space portrait is shown in Fig. 5. Where the classical particle is a point on an orbit, the quantum particle is spread out, obeying the Δx-Δp Heisenberg product, but following the same orbit as the classical particle.
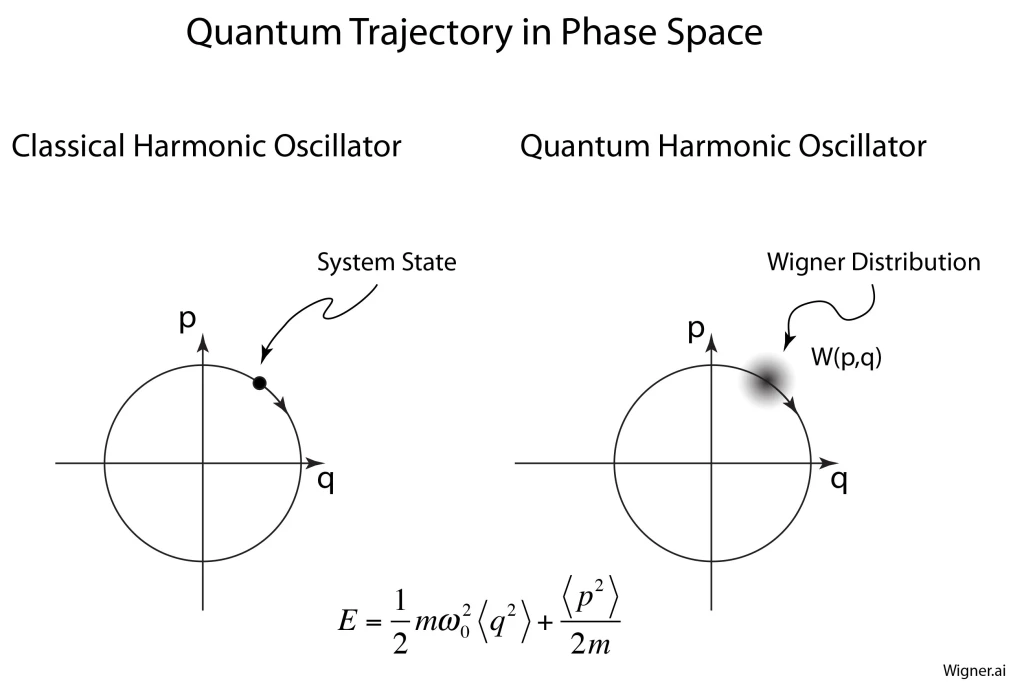
However, a significant new feature appears in the Wigner representation in phase space when there is a coherent superposition of two states, known as a “cat” state, after Schrödinger’s cat. This new feature has no classical analog. It is the coherent interference pattern that appears at the zero-point of the harmonic oscillator for the Schrödinger cat state. There is no such thing as “classical” coherence, so this feature is absent in classical phase space portraits.
Two examples of Wigner distributions are shown in Fig. 6 for a statistical (incoherent) mixture of packets and a coherent superposition of packets. The quantum coherence signature is present in the coherent case but not the statistical mixture case. The coherence in the Wigner distribution represents “off-diagonal” terms in the density matrix that leads to interference effects in quantum systems. Quantum computing algorithms depend critically on such coherences that tend to decay rapidly in real-world physical systems, known as decoherence, and it is possible to make statements about decoherence by watching the zero-point interference.

Whereas Gaussian wave packets in the quantum harmonic potential behave nearly like classical systems, and their phase-space portraits are almost identical to the classical phase-space view (except for the quantum coherence), most quantum potentials cause wave packets to disperse. And when saddle points are present in the classical case, then we are back to the question about how quantum packets behave as they approach a saddle point in phase space.
Quantum Pendulum and Separatrix Chaos
One of the simplest anharmonic oscillators is the simple pendulum. In the classical case, the period diverges if the pendulum gets very close to going vertical. A similar thing happens in the quantum case, but because the motion has strong anharmonicity, an initial wave packet tends to spread dramatically as parts of the wavefunction less vertical stretch away from the part of the wave function that is more nearly vertical. Fig. 7 is a snap-shot about a eighth of a period after the wave packet was launched. The packet has already stretched out along the separatrix. A double-cat-state was used, so there is a second packet that has coherent interference with the first. To see a movie of the time evolution of the wave packet and the orbit in quantum phase space, see the YouTube video.

The simple pendulum does have a saddle point, but it is degenerate because the angle is modulo -2-pi. A simple potential that has a non-degenerate saddle point is a double-well potential.
Quantum Double-Well and Separatrix Chaos
The symmetric double-well potential has a saddle point at the mid-point between the two well minima. A wave packet approaching the saddle will split into to packets that will follow the individual separatrixes that emerge from the saddle point (the unstable manifolds). This effect is seen most dramatically in the middle pane of Fig. 8. For the full video of the quantum phase-space evolution, see this YouTube video. The stretched-out distribution in phase space is highly analogous to the separatrix chaos seen for the classical system.

Conclusion
A common statement often made about quantum chaos is that quantum systems tend to suppress chaos, only exhibiting chaos for special types of orbits that produce quantum scars. However, from the phase-space perspective, the opposite may be true. The stretched-out Wigner distribution functions, for critical wave packets that interact with a saddle point, are very similar to the stochastic layer that forms in separatrix chaos in classical systems. In this sense, the phase-space description brings out the similarity between classical chaos and quantum chaos.
By David D. Nolte Sept. 25, 2022
YouTube Video
For more on the history of quantum trajectories, see Galileo Unbound from Oxford Press:
References
1. T. Curtright, D. Fairlie, C. Zachos, A Concise Treatise on Quantum Mechanics in Phase Space. (World Scientific, New Jersey, 2014).
2. J. R. Nagel, A Review and Application of the Finite-Difference Time-Domain Algorithm Applied to the Schrödinger Equation, ACES Journal, Vol. 24, NO. 1, pp. 1-8 (2009)