Light is one of the most powerful manifestations of the forces of physics because it tells us about our reality. The interference of light, in particular, has led to the detection of exoplanets orbiting distant stars, discovery of the first gravitational waves, capture of images of black holes and much more. The stories behind the history of light and interference go to the heart of how scientists do what they do and what they often have to overcome to do it. These time-lines are organized along the chapter titles of the book Interference. They follow the path of theories of light from the first wave-particle debate, through the personal firestorms of Albert Michelson, to the discoveries of the present day in quantum information sciences.
- Thomas Young Polymath: The Law of Interference
- The Fresnel Connection: Particles versus Waves
- At Light Speed: The Birth of Interferometry
- After the Gold Rush: The Trials of Albert Michelson
- Stellar Interference: Measuring the Stars
- Across the Universe: Exoplanets, Black Holes and Gravitational Waves
- Two Faces of Microscopy: Diffraction and Interference
- Holographic Dreams of Princess Leia: Crossing Beams
- Photon Interference: The Foundations of Quantum Communication
- The Quantum Advantage: Interferometric Computing
1. Thomas Young Polymath: The Law of Interference
Thomas Young was the ultimate dabbler, his interests and explorations ranged far and wide, from ancient egyptology to naval engineering, from physiology of perception to the physics of sound and light. Yet unlike most dabblers who accomplish little, he made original and seminal contributions to all these fields. Some have called him the “Last Man Who Knew Everything“.
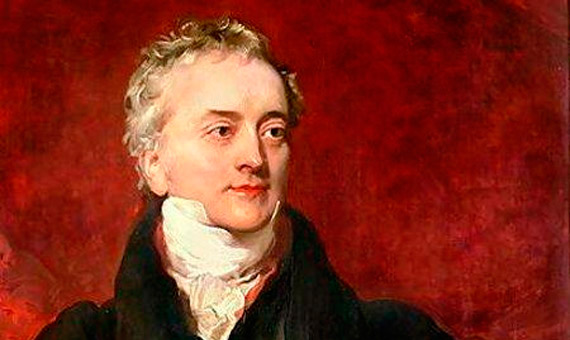
Topics: The Law of Interference. The Rosetta Stone. Benjamin Thompson, Count Rumford. Royal Society. Christiaan Huygens. Pendulum Clocks. Icelandic Spar. Huygens’ Principle. Stellar Aberration. Speed of Light. Double-slit Experiment.
1629 – Huygens born (1629 – 1695)
1642 – Galileo dies, Newton born (1642 – 1727)
1655 – Huygens ring of Saturn
1657 – Huygens patents the pendulum clock
1666 – Newton prismatic colors
1666 – Huygens moves to Paris
1669 – Bartholin double refraction in Icelandic spar
1670 – Bartholinus polarization of light by crystals
1671 – Expedition to Hven by Picard and Rømer
1673 – James Gregory bird-feather diffraction grating
1673 – Huygens publishes Horologium Oscillatorium
1675 – Rømer finite speed of light
1678 – Huygens and two crystals of Icelandic spar
1681 – Huygens returns to the Hague
1689 – Huyens meets Newton
1690 – Huygens Traite de la Lumiere
1695 – Huygens dies
1704 – Newton’s Opticks
1727 – Bradley abberation of starlight
1746 – Euler Nova theoria lucis et colorum
1773 – Thomas Young born
1786 – François Arago born (1786 – 1853)
1787 – Joseph Fraunhofer born (1787 – 1826)
1788 – Fresnel born in Broglie, Normandy (1788 – 1827)
1794 – École Polytechnique founded in Paris by Lazar Carnot and Gaspard Monge, Malus enters the Ecole
1794 – Young elected member of the Royal Society
1794 – Young enters Edinburg (cannot attend British schools because he was Quaker)
1795 – Young enters Göttingen
1796 – Young receives doctor of medicine, grand tour of Germany
1797 – Young returns to England, enters Emmanual College (converted to Church of England)
1798 – The Directory approves Napoleon’s Egyptian campaign, Battle of the Pyramids, Battle of the Nile
1799 – Young graduates from Cambridge
1799 – Royal Institution founded
1799 – Young Outlines
1800 – Young Sound and Light read to Royal Society,
1800 – Young Mechanisms of the Eye (Bakerian Lecture of the Royal Society)
1801 – Young Theory of Light and Colours, three color mechanism (Bakerian Lecture), Young considers interference to cause the colored films, first estimates of the wavelengths of different colors
1802 – Young begins series of lecturs at the Royal Institution (Jan. 1802 – July 1803)
1802 – Young names the principle (Law) of interference
1803 – Young’s 3rd Bakerian Lecture, November. Experiments and Calculations Relative Physical to Optics, The Law of Interference
1807 – Young publishes A course of lectures on Natural Philosophy and the Mechanical Arts, based on Royal Institution lectures, two-slit experiment described
1808 – Malus polarization
1811 – Young appointed to St. Georges hospital
1813 – Young begins work on Rosetta stone
1814 – Young translates the demotic script on the stone
1816 – Arago visits Young
1818 – Young’s Encyclopedia article on Egypt
1822 – Champollion publishes translation of hieroglyphics
1827 – Young elected foreign member of the Institute of Paris
1829 – Young dies
2. The Fresnel Connection: Particles versus Waves
Augustin Fresnel was an intuitive genius whose talents were almost squandered on his job building roads and bridges in the backwaters of France until he was discovered and rescued by Francois Arago.
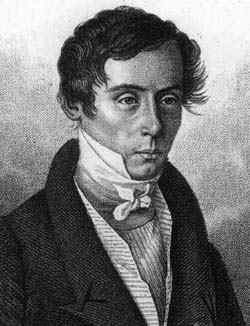
Topics: Particles versus Waves. Malus and Polarization. Agustin Fresnel. Francois Arago. Diffraction. Daniel Bernoulli. The Principle of Superposition. Joseph Fourier. Transverse Light Waves.
1665 – Grimaldi diffraction bands outside shadow
1673 – James Gregory bird-feather diffraction grating
1675 – Römer finite speed of light
1704 – Newton’s Optics
1727 – Bradley abberation of starlight
1774 – Jean-Baptiste Biot born
1786 – David Rittenhouse hairs-on-screws diffraction grating
1786 – François Arago born (1786 – 1853)
1787 – Fraunhofer born (1787 – 1826)
1788 – Fresnel born in Broglie, Normandy (1788 – 1827)
1790 – Fresnel moved to Cherbourg
1794 – École Polytechnique founded in Paris by Lazar Carnot and Gaspard Monge
1804 – Fresnel attends Ecole polytechnique in Paris at age 16
1806 – Fresnel graduated and attended the national school of bridges and highways
1808 – Malus polarization
1809 – Fresnel graduated from Les Ponts
1809 – Arago returns from captivity in Algiers
1811 – Arago publishes paper on particle theory of light
1811 – Arago optical ratotory activity (rotation)
1814 – Fraunhofer spectroscope (solar absorption lines)
1815 – Fresnel meets Arago in Paris on way home to Mathieu (for house arrest)
1815 – Fresnel first paper on wave properties of diffraction
1816 – Fresnel returns to Paris to demonstrate his experiments
1816 – Arago visits Young
1816 – Fresnel paper on interference as origin of diffraction
1817 – French Academy announces its annual prize competition: topic of diffraction
1817 – Fresnel invents and uses his “Fresnel Integrals”
1819 – Fresnel awarded French Academy prize for wave theory of diffraction
1819 – Arago and Fresnel transverse and circular (?) polarization
1821 – Fraunhofer diffraction grating
1821 – Fresnel light is ONLY transverse
1821 – Fresnel double refraction explanation
1823 – Fraunhofer 3200 lines per Paris inch
1826 – Publication of Fresnel’s award memoire
1827 – Death of Fresnel by tuberculosis
1840 – Ernst Abbe born (1840 – 1905)
1849 – Stokes distribution of secondary waves
1850 – Fizeau and Foucault speed of light experiments
3. At Light Speed
There is no question that Francois Arago was a swashbuckler. His life’s story reads like an adventure novel as he went from being marooned in hostile lands early in his career to becoming prime minister of France after the 1848 revolutions swept across Europe.
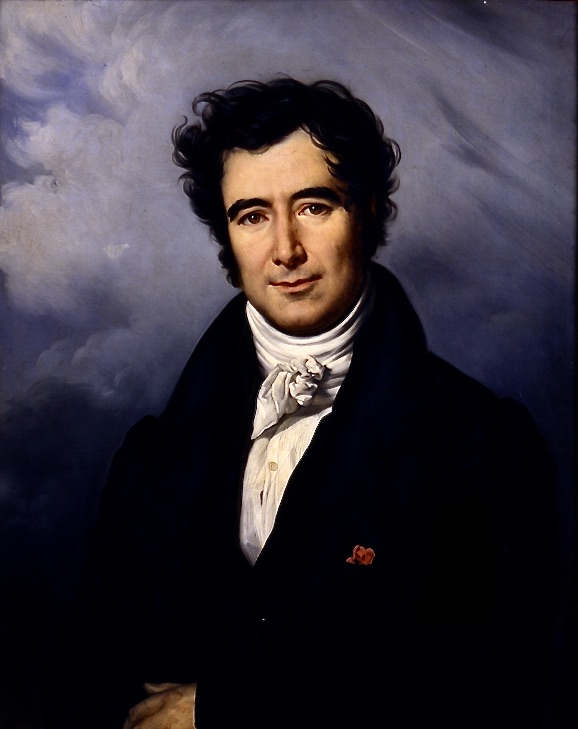
Topics: The Birth of Interferometry. Snell’s Law. Fresnel and Arago. The First Interferometer. Fizeau and Foucault. The Speed of Light. Ether Drag. Jamin Interferometer.
1671 – Expedition to Hven by Picard and Rømer
1704 – Newton’s Opticks
1729 – James Bradley observation of stellar aberration
1784 – John Michel dark stars
1804 – Young wave theory of light and ether
1808 – Malus discovery of polarization of reflected light
1810 – Arago search for ether drag
1813 – Fraunhofer dark lines in Sun spectrum
1819 – Fresnel’s double mirror
1820 – Oersted discovers electromagnetism
1821 – Faraday electromagnetic phenomena
1821 – Fresnel light purely transverse
1823 – Fresnel reflection and refraction based on boundary conditions of ether
1827 – Green mathematical analysis of electricity and magnetism
1830 – Cauchy ether as elastic solid
1831 – Faraday electromagnetic induction
1831 – Cauchy ether drag
1831 – Maxwell born
1831 – Faraday electromagnetic induction
1834 – Lloyd’s mirror
1836 – Cauchy’s second theory of the ether
1838 – Green theory of the ether
1839 – Hamilton group velocity
1839 – MacCullagh properties of rotational ether
1839 – Cauchy ether with negative compressibility
1841 – Maxwell entered Edinburgh Academy (age 10) met P. G. Tait
1842 – Doppler effect
1845 – Faraday effect (magneto-optic rotation)
1846 – Haidinger fringes
1846 – Stokes’ viscoelastic theory of the ether
1847 – Maxwell entered Edinburgh University
1848 – Fizeau proposal of the Fizeau-Doppler effect
1849 – Fizeau speed of light
1850 – Maxwell at Cambridge, studied under Hopkins, also knew Stokes and Whewell
1852 – Michelson born Strelno, Prussia
1854 – Maxwell wins the Smith’s Prize (Stokes’ theorem was one of the problems)
1855 – Michelson’s immigrate to San Francisco through Panama Canal
1855 – Maxwell “On Faraday’s Line of Force”
1856 – Jamin interferometer
1856 – Thomson magneto-optics effects (of Faraday)
1857 – Clausius constructs kinetic theory, Mean molecular speeds
1859 – Fizeau light in moving medium
1862 – Fizeau fringes
1865 – Maxwell “A Dynamical Theory of the Electromagnetic Field”
1867 – Thomson and Tait “Treatise on Natural Philosophy”
1867 – Thomson hydrodynamic vortex atom
1868 – Fizeau proposal for stellar interferometry
1870 – Maxwell introduced “curl”, “convergence” and “gradient”
1871 – Maxwell appointed to Cambridge
1873 – Maxwell “A Treatise on Electricity and Magnetism”
4. After the Gold Rush
No name is more closely connected to interferometry than that of Albert Michelson. He succeeded, sometimes at great personal cost, in launching interferometric metrology as one of the most important tools used by scientists today.

Topics: The Trials of Albert Michelson. Hermann von Helmholtz. Michelson and Morley. Fabry and Perot.
1810 – Arago search for ether drag
1813 – Fraunhofer dark lines in Sun spectrum
1813 – Faraday begins at Royal Institution
1820 – Oersted discovers electromagnetism
1821 – Faraday electromagnetic phenomena
1827 – Green mathematical analysis of electricity and magnetism
1830 – Cauchy ether as elastic solid
1831 – Faraday electromagnetic induction
1831 – Cauchy ether drag
1831 – Maxwell born
1831 – Faraday electromagnetic induction
1836 – Cauchy’s second theory of the ether
1838 – Green theory of the ether
1839 – Hamilton group velocity
1839 – MacCullagh properties of rotational ether
1839 – Cauchy ether with negative compressibility
1841 – Maxwell entered Edinburgh Academy (age 10) met P. G. Tait
1842 – Doppler effect
1845 – Faraday effect (magneto-optic rotation)
1846 – Stokes’ viscoelastic theory of the ether
1847 – Maxwell entered Edinburgh University
1850 – Maxwell at Cambridge, studied under Hopkins, also knew Stokes and Whewell
1852 – Michelson born Strelno, Prussia
1854 – Maxwell wins the Smith’s Prize (Stokes’ theorem was one of the problems)
1855 – Michelson’s immigrate to San Francisco through Panama Canal
1855 – Maxwell “On Faraday’s Line of Force”
1856 – Jamin interferometer
1856 – Thomson magneto-optics effects (of Faraday)
1859 – Fizeau light in moving medium
1859 – Discovery of the Comstock Lode
1860 – Maxwell publishes first paper on kinetic theory.
1861 – Maxwell “On Physical Lines of Force” speed of EM waves and molecular vortices, molecular vortex model
1862 – Michelson at boarding school in SF
1865 – Maxwell “A Dynamical Theory of the Electromagnetic Field”
1867 – Thomson and Tait “Treatise on Natural Philosophy”
1867 – Thomson hydrodynamic vortex atom
1868 – Fizeau proposal for stellar interferometry
1869 – Michelson meets US Grant and obtained appointment to Annapolis
1870 – Maxwell introduced “curl”, “convergence” and “gradient”
1871 – Maxwell appointed to Cambridge
1873 – Big Bonanza at the Consolidated Virginia mine
1873 – Maxwell “A Treatise on Electricity and Magnetism”
1873 – Michelson graduates from Annapolis
1875 – Michelson instructor at Annapolis
1877 – Michelson married Margaret Hemingway
1878 – Michelson First measurement of the speed of light with funds from father in law
1879 – Michelson Begin collaborating with Newcomb
1879 – Maxwell proposes second-order effect for ether drift experiments
1879 – Maxwell dies
1880 – Michelson Idea for second-order measurement of relative motion against ether
1880 – Michelson studies in Europe with Helmholtz in Berlin
1881 – Michelson Measurement at Potsdam with funds from Alexander Graham Bell
1882 – Michelson in Paris, Cornu, Mascart and Lippman
1882 – Michelson Joined Case School of Applied Science
1884 – Poynting energy flux vector
1885 – Michelson Began collaboration with Edward Morley of Western Reserve
1885 – Lorentz points out inconsistency of Stokes’ ether model
1885 – Fitzgerald wheel and band model, vortex sponge
1886 – Michelson and Morley repeat the Fizeau moving water experiment
1887 – Michelson Five days in July experiment on motion relative to ether
1887 – Michelson-Morley experiment published
1887 – Voigt derivation of relativistic Doppler (with coordinate transformations)
1888 – Hertz generation and detection of radio waves
1889 – Michelson moved to Clark University at Worcester
1889 – Fitzgerald contraction
1889 – Lodge cogwheel model of electromagnetism
1890 – Michelson Proposed use of interferometry in astronomy
1890 – Thomson devises a mechanical model of MacCullagh’s rotational ether
1890 – Hertz Galileo relativity and ether drag
1891 – Mach-Zehnder
1891 – Michelson measures diameter of Jupiter’s moons with interferometry
1891 – Thomson vortex electromagnetism
1892 – 1893 Michelson measurement of the Paris meter
1893 – Sirks interferometer
1893 – Michelson moved to University of Chicago to head Physics Dept.
1893 – Lorentz contraction
1894 – Lodge primitive radio demonstration
1895 – Marconi radio
1896 – Rayleigh’s interferometer
1897 – Lodge no ether drag on laboratory scale
1898 – Pringsheim interferometer
1899 – Fabry-Perot interferometer
1899 – Michelson remarried
1901 – 1903 Michelson President of the APS
1905 – Poincaré names the Lorentz transformations
1905 – Einstein’s special theory of Relativity
1907 – Michelson Nobel Prize
1913 – Sagnac interferometer
1916 – Twyman-Green interferometer
1920 – Stellar interferometer on the Hooker 100-inch telescope (Betelgeuse)
1923 – 1927 Michelson presided over the National Academy of Sciences
1931 – Michelson dies
5. Stellar Interference
Learning from his attempts to measure the speed of light through the ether, Michelson realized that the partial coherence of light from astronomical sources could be used to measure their sizes. His first measurements using the Michelson Stellar Interferometer launched a major subfield of astronomy that is one of the most active today.

Topics: Measuring the Stars. Astrometry. Moons of Jupiter. Schwarzschild. Betelgeuse. Michelson Stellar Interferometer. Banbury Brown Twiss. Sirius. Adaptive Optics.
1838 – Bessel stellar parallax measurement with Fraunhofer telescope
1868 – Fizeau proposes stellar interferometry
1873 – Stephan implements Fizeau’s stellar interferometer on Sirius, sees fringes
1880 – Michelson Idea for second-order measurement of relative motion against ether
1880 – 1882 Michelson Studies in Europe (Helmholtz in Berlin, Quincke in Heidelberg, Cornu, Mascart and Lippman in Paris)
1881 – Michelson Measurement at Potsdam with funds from Alexander Graham Bell
1881 – Michelson Resigned from active duty in the Navy
1883 – Michelson Joined Case School of Applied Science
1889 – Michelson moved to Clark University at Worcester
1890 – Michelson develops mathematics of stellar interferometry
1891 – Michelson measures diameters of Jupiter’s moons
1893 – Michelson moves to University of Chicago to head Physics Dept.
1896 – Schwarzschild double star interferometry
1907 – Michelson Nobel Prize
1908 – Hale uses Zeeman effect to measure sunspot magnetism
1910 – Taylor single-photon double slit experiment
1915 – Proxima Centauri discovered by Robert Innes
1916 – Einstein predicts gravitational waves
1920 – Stellar interferometer on the Hooker 100-inch telescope (Betelgeuse)
1947 – McCready sea interferometer observes rising sun (first fringes in radio astronomy
1952 – Ryle radio astronomy long baseline
1954 – Hanbury-Brown and Twiss radio intensity interferometry
1956 – Hanbury-Brown and Twiss optical intensity correlation, Sirius (optical)
1958 – Jennison closure phase
1970 – Labeyrie speckle interferometry
1974 – Long-baseline radio interferometry in practice using closure phase
1974 – Johnson, Betz and Townes: IR long baseline
1975 – Labeyrie optical long-baseline
1982 – Fringe measurements at 2.2 microns Di Benedetto
1985 – Baldwin closure phase at optical wavelengths
1991 – Coude du Foresto single-mode fibers with separated telescopes
1993 – Nobel prize to Hulse and Taylor for binary pulsar
1995 – Baldwin optical synthesis imaging with separated telescopes
1991 – Mayor and Queloz Doppler pull of 51 Pegasi
1999 – Upsilon Andromedae multiple planets
2009 – Kepler space telescope launched
2014 – Kepler announces 715 planets
2015 – Kepler-452b Earthlike planet in habitable zone
2015 – First detection of gravitational waves
2016 – Proxima Centauri b exoplanet confirmed
2017 – Nobel prize for gravitational waves
2018 – TESS (Transiting Exoplanet Survey Satellite)
2019 – Mayor and Queloz win Nobel prize for first exoplanet
2019 – First direct observation of exoplanet using interferometry
2019 – First image of a black hole obtained by very-long-baseline interferometry
6. Across the Universe
Stellar interferometry is opening new vistas of astronomy, exploring the wildest occupants of our universe, from colliding black holes half-way across the universe (LIGO) to images of neighboring black holes (EHT) to exoplanets near Earth that may harbor life.
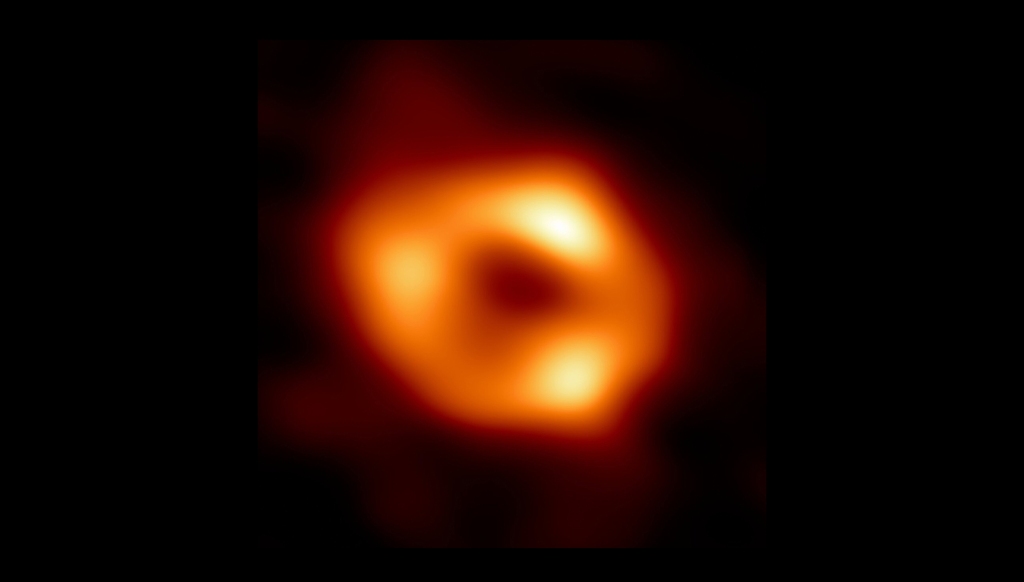
Topics: Gravitational Waves, Black Holes and the Search for Exoplanets. Nulling Interferometer. Event Horizon Telescope. M87 Black Hole. Long Baseline Interferometry. LIGO.
1947 – Virgo A radio source identified as M87
1953 – Horace W. Babcock proposes adaptive optics (AO)
1958 – Jennison closure phase
1967 – First very long baseline radio interferometers (from meters to hundreds of km to thousands of km within a single year)
1967 – Ranier Weiss begins first prototype gravitational wave interferometer
1967 – Virgo X-1 x-ray source (M87 galaxy)
1970 – Poul Anderson’s Tau Zero alludes to AO in science fiction novel
1973 – DARPA launches adaptive optics research with contract to Itek, Inc.
1974 – Wyant (Itek) white-light shearing interferometer
1974 – Long-baseline radio interferometry in practice using closure phase
1975 – Hardy (Itek) patent for adaptive optical system
1975 – Weiss funded by NSF to develop interferometer for GW detection
1977 – Demonstration of AO on Sirius (Bell Labs and Berkeley)
1980 – Very Large Array (VLA) 6 mm to 4 meter wavelengths
1981 – Feinleib proposes atmospheric laser backscatter
1982 – Will Happer at Princeton proposes sodium guide star
1982 – Fringe measurements at 2.2 microns (Di Benedetto)
1983 – Sandia Optical Range demonstrates artificial guide star (Rayleigh)
1983 – Strategic Defense Initiative (Star Wars)
1984 – Lincoln labs sodium guide star demo
1984 – ESO plans AO for Very Large Telescope (VLT)
1985 – Laser guide star (Labeyrie)
1985 – Closure phase at optical wavelengths (Baldwin)
1988 – AFWL names Starfire Optical Range, Kirtland AFB outside Albuquerque
1988 – Air Force Maui Optical Site Schack-Hartmann and 241 actuators (Itek)
1988 – First funding for LIGO feasibility
1989 – 19-element-mirror Double star on 1.5m telescope in France
1989 – VLT approved for construction
1990 – Launch of the Hubble Space Telescope
1991 – Single-mode fibers with separated telescopes (Coude du Foresto)
1992 – ADONIS
1992 – NSF requests declassification of AO
1993 – VLBA (Very Long Baseline Array) 8,611 km baseline 3 mm to 90 cm
1994 – Declassification completed
1994 – Curvature sensor 3.6m Canada-France-Hawaii
1994 – LIGO funded by NSF, Barish becomes project director
1995 – Optical synthesis imaging with separated telescopes (Baldwin)
1995 – Doppler pull of 51 Pegasi (Mayor and Queloz)
1998 – ESO VLT first light
1998 – Keck installed with Schack-Hartmann
1999 – Upsilon Andromedae multiple planets
2000 – Hale 5m Palomar Schack-Hartmann
2001 – NAOS-VLT adaptive optics
2001 – VLTI first light (MIDI two units)
2002 – LIGO operation begins
2007 – VLT laser guide star
2007 – VLTI AMBER first scientific results (3 units)
2009 – Kepler space telescope launched
2009 – Event Horizon Telescope (EHT) project starts
2010 – Large Binocular Telescope (LBT) 672 actuators on secondary mirror
2010 – End of first LIGO run. No events detected. Begin Enhanced LIGO upgrade.
2011 – SPHERE-VLT 41×41 actuators (1681)
2012 – Extremely Large Telescope (ELT) approved for construction
2014 – Kepler announces 715 planets
2015 – Kepler-452b Earthlike planet in habitable zone
2015 – First detection of gravitational waves (LIGO)
2015 – LISA Pathfinder launched
2016 – Second detection at LIGO
2016 – Proxima Centauri b exoplanet confirmed
2016 – GRAVITY VLTI (4 units)
2017 – Nobel prize for gravitational waves
2018 – TESS (Transiting Exoplanet Survey Satellite) launched
2018 – MATTISE VLTI first light (combining all units)
2019 – Mayor and Queloz win Nobel prize
2019 – First direct observation of exoplanet using interferometry at LVTI
2019 – First image of a black hole obtained by very-long-baseline interferometry (EHT)
2020 – First neutron-star black-hole merger detected
2020 – KAGRA (Japan) online
2024 – LIGO India to go online
2025 – First light for ELT
2034 – Launch date for LISA
7. Two Faces of Microscopy
From the astronomically large dimensions of outer space to the microscopically small dimensions of inner space, optical interference pushes the resolution limits of imaging.
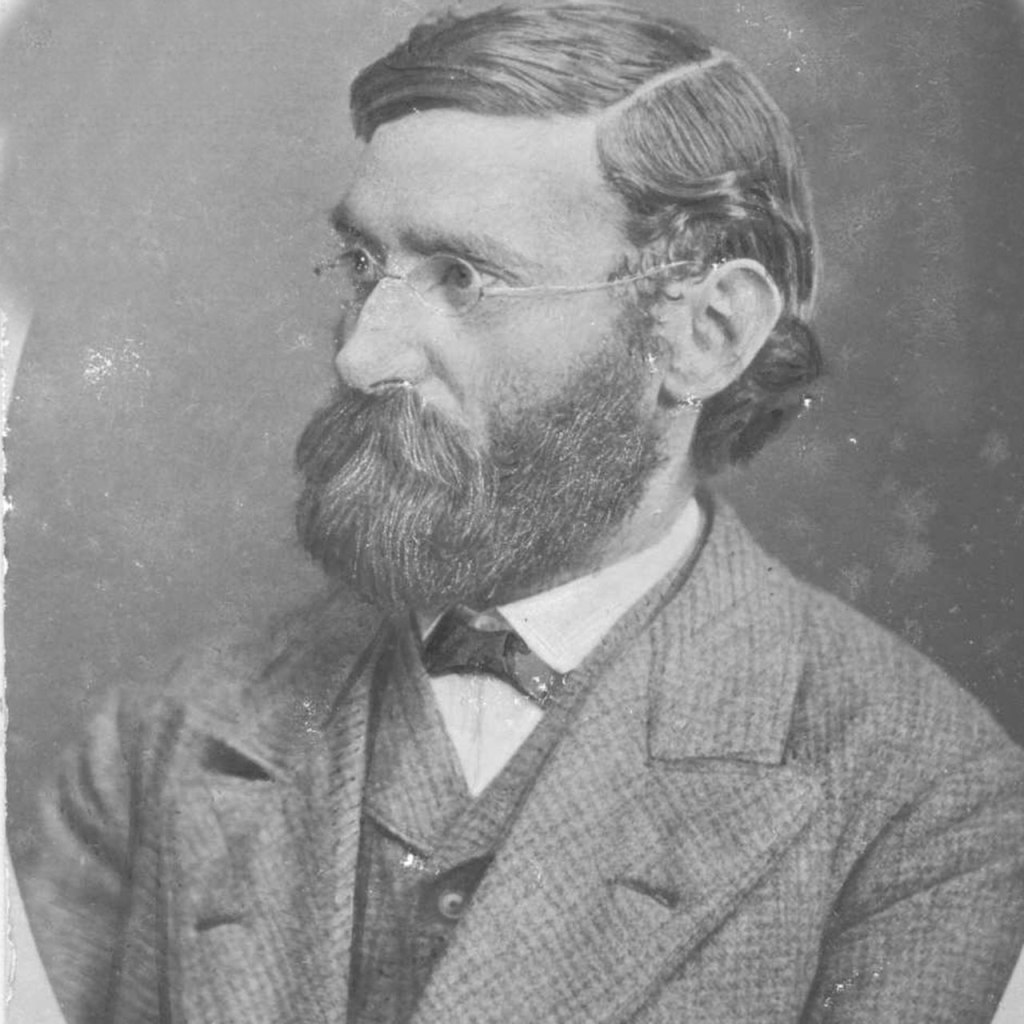
Topics: Diffraction and Interference. Joseph Fraunhofer. Diffraction Gratings. Henry Rowland. Carl Zeiss. Ernst Abbe. Phase-contrast Microscopy. Super-resolution Micrscopes. Structured Illumination.
1021 – Al Hazeni manuscript on Optics
1284 – First eye glasses by Salvino D’Armate
1590 – Janssen first microscope
1609 – Galileo first compound microscope
1625 – Giovanni Faber coins phrase “microscope”
1665 – Hook’s Micrographia
1676 – Antonie van Leeuwenhoek microscope
1787 – Fraunhofer born
1811 – Fraunhofer enters business partnership with Utzschneider
1816 – Carl Zeiss born
1821 – Fraunhofer first diffraction publication
1823 – Fraunhofer second diffraction publication 3200 lines per Paris inch
1830 – Spherical aberration compensated by Joseph Jackson Lister
1840 – Ernst Abbe born
1846 – Zeiss workshop in Jena, Germany
1850 – Fizeau and Foucault speed of light
1851 – Otto Schott born
1859 – Kirchhoff and Bunsen theory of emission and absorption spectra
1866 – Abbe becomes research director at Zeiss
1874 – Ernst Abbe equation on microscope resolution
1874 – Helmholtz image resolution equation
1880 – Rayleigh resolution
1888 – Hertz waves
1888 – Frits Zernike born
1925 – Zsigmondy Nobel Prize for light-sheet microscopy
1931 – Transmission electron microscope by Ruske and Knoll
1932 – Phase contrast microscope by Zernicke
1942 – Scanning electron microscope by Ruska
1949 – Mirau interferometric objective
1952 – Nomarski differential phase contrast microscope
1953 – Zernicke Nobel prize
1955 – First discussion of superresolution by Toraldo di Francia
1957 – Marvin Minsky patents confocal principle
1962 – Green flurescence protein (GFP) Shimomura, Johnson and Saiga
1966 – Structured illumination microscopy by Lukosz
1972 – CAT scan
1978 – Cremer confocal laser scanning microscope
1978 – Lohman interference microscopy
1981 – Binnig and Rohrer scanning tunneling microscope (STM)
1986 – Microscopy Nobel Prize: Ruska, Binnig and Rohrer
1990 – 4PI microscopy by Stefan Hell
1992 – GFP cloned
1993 – STED by Stefan Hell
1993 – Light sheet fluorescence microscopy by Spelman
1995 – Structured illumination microscopy by Guerra
1995 – Gustafsson image interference microscopy
1999 – Gustafsson I5M
2004 – Selective plane illumination microscopy (SPIM)
2006 – PALM and STORM (Betzig and Zhuang)
2014 – Nobel Prize (Hell, Betzig and Moerner)
8. Holographic Dreams of Princess Leia
The coherence of laser light is like a brilliant jewel that sparkles in the darkness, illuminating life, probing science and projecting holograms in virtual worlds.

Topics: Crossing Beams. Denis Gabor. Wavefront Reconstruction. Holography. Emmett Leith. Lasers. Ted Maiman. Charles Townes. Optical Maser. Dynamic Holography. Light-field Imaging.
1900 – Dennis Gabor born
1926 – Hans Busch magnetic electron lens
1927 – Gabor doctorate
1931 – Ruska and Knoll first two-stage electron microscope
1942 – Lawrence Bragg x-ray microscope
1948 – Gabor holography paper in Nature
1949 – Gabor moves to Imperial College
1950 – Lamb possibility of population inversion
1951 – Purcell and Pound demonstration of population inversion
1952 – Leith joins Willow Run Labs
1953 – Townes first MASER
1957 – SAR field trials
1957 – Gould coins LASER
1958 – Schawlow and Townes proposal for optical maser
1959 – Shawanga Lodge conference
1960 – Maiman first laser: pink ruby
1960 – Javan first gas laser: HeNe at 1.15 microns
1961 – Leith and Upatnieks wavefront reconstruction
1962 – HeNe laser in the visible at 632.8 nm
1962 – First laser holograms (Leith and Upatnieks)
1963 – van Heerden optical information storage
1963 – Leith and Upatnieks 3D holography
1966 – Ashkin optically-induced refractive index changes
1966 – Leith holographic information storage in 3D
1968 – Bell Labs holographic storage in Lithium Niobate and Tantalate
1969 – Kogelnik coupled wave theory for thick holograms
1969 – Electrical control of holograms in SBN
1970 – Optically induced refractive index changes in Barium Titanate
1971 – Amodei transport models of photorefractive effect
1971 – Gabor Nobel prize
1972 – Staebler multiple holograms
1974 – Glass and von der Linde photovoltaic and photorefractive effects, UV erase
1977 – Star Wars movie
1981 – Huignard two-wave mixing energy transfer
2012 – Coachella Music Festival
9. Photon Interference
What is the image of one photon interfering? Better yet, what is the image of two photons interfering? The answer to this crucial question laid the foundation for quantum communication.

Topics: The Beginnings of Quantum Communication. EPR paradox. Entanglement. David Bohm. John Bell. The Bell Inequalities. Leonard Mandel. Single-photon Interferometry. HOM Interferometer. Two-photon Fringes. Quantum cryptography. Quantum Teleportation.
1900 – Planck (1901). “Law of energy distribution in normal spectra.” [1]
1905 – A. Einstein (1905). “Generation and conversion of light wrt a heuristic point of view.” [2]
1909 – A. Einstein (1909). “On the current state of radiation problems.” [3]
1909 – Single photon double-slit experiment, G.I. Taylor [4]
1915 – Milliken photoelectric effect
1916 – Einstein predicts stimulated emission
1923 –Compton, Arthur H. (May 1923). Quantum Theory of the Scattering of X-Rays.[5]
1926 – Gilbert Lewis names “photon”
1926 – Dirac: photons interfere only with themselves
1927 – D. Dirac, P. A. M. (1927). Emission and absorption of radiation [6]
1932 – von Neumann textbook on quantum physics
1932 – E. P. Wigner: Phys. Rev. 40, 749 (1932)
1935 – EPR paper, A. Einstein, B. Podolsky, N. Rosen: Phys. Rev. 47 , 777 (1935)
1935 – Reply to EPR, N. Bohr: Phys. Rev. 48 , 696 (1935)
1935 – Schrödinger (1935 and 1936) on entanglement (cat?) “Present situation in QM”
1948 – Gabor holography
1950 – Wu correlated spin generation from particle decay
1951 – Bohm alternative form of EPR gedankenexperiment (quantum textbook)
1952 – Bohm nonlocal hidden variable theory[7]
1953 – Schwinger: Coherent states
1956 – Photon bunching, R. Hanbury-Brown, R.W. Twiss: Nature 177 , 27 (1956)
1957 – Bohm and Ahronov proof of entanglement in 1950 Wu experiment
1959 – Ahronov-Bohm effect of magnetic vector potential
1960 – Klauder: Coherent states
1963 – Coherent states, R. J. Glauber: Phys. Rev. 130 , 2529 (1963)
1963 – Coherent states, E. C. G. Sudarshan: Phys. Rev. Lett. 10, 277 (1963)
1964 – J. S. Bell: Bell inequalities [8]
1964 – Mandel professorship at Rochester
1967 – Interference at single photon level, R. F. Pfleegor, L. Mandel: [9]
1967 – M. O. Scully, W.E. Lamb: Phys. Rev. 159 , 208 (1967) Quantum theory of laser
1967 – Parametric converter (Mollow and Glauber) [10]
1967 – Kocher and Commins calcium 2-photon cascade
1969 – Quantum theory of laser, M. Lax, W.H. Louisell: Phys. Rev. 185 , 568 (1969)
1969 – CHSH inequality [11]
1972 – First test of Bell’s inequalities (Freedman and Clauser)
1975 – Carmichel and Walls predicted light in resonance fluorescence from a two-level atom would display photon anti-bunching (1976)
1977 – Photon antibunching in resonance fluorescence. H. J. Kimble, M. Dagenais and L. Mandel [12]
1978 – Kip Thorne quantum non-demolition (QND)
1979 – Hollenhorst squeezing for gravitational wave detection: names squeezing
1982 – Apect Experimental Bell experiments, [13]
1985 – Dick Slusher experimental squeezing
1985 – Deutsch quantum algorithm
1986 – Photon anti-bunching at a beamsplitter, P. Grangier, G. Roger, A. Aspect: [14]
1986 – Kimble squeezing in parametric down-conversion
1986 – C. K. Hong, L. Mandel: Phys. Rev. Lett. 56 , 58 (1986) one-photon localization
1987 – Two-photon interference (Ghosh and Mandel) [15]
1987 – HOM effect [16]
1987 – Photon squeezing, P. Grangier, R. E. Slusher, B. Yurke, A. La Porta: [17]
1987 – Grangier and Slusher, squeezed light interferometer
1988 – 2-photon Bell violation: Z. Y. Ou, L. Mandel: Phys. Rev. Lett. 61 , 50 (1988)
1988 – Brassard Quantum cryptography
1989 – Franson proposes two-photon interference in k-number (?)
1990 – Two-photon interference in k-number (Kwiat and Chiao)
1990 – Two-photon interference (Ou, Zhou, Wang and Mandel)
1993 – Quantum teleportation proposal (Bennett)
1994 – Teleportation of quantum states (Vaidman)
1994 – Shor factoring algorithm
1995 – Down-conversion for polarization: Kwiat and Zeilinger (1995)
1997 – Experimental quantum teleportation (Bouwmeester)
1997 – Experimental quantum teleportation (Bosci)
1998 – Unconditional quantum teleportation (every state) (Furusawa)
2001 – Quantum computing with linear optics (Knill, Laflamme, Milburn)
2013 – LIGO design proposal with squeezed light (Aasi)
2019 – Squeezing upgrade on LIGO (Tse)
2020 – Quantum computational advantage (Zhong)
10. The Quantum Advantage
There is almost no technical advantage better than having exponential resources at hand. The exponential resources of quantum interference provide that advantage to quantum computing which is poised to usher in a new era of quantum information science and technology.
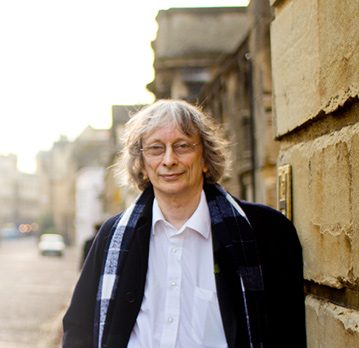
Topics: Interferometric Computing. David Deutsch. Quantum Algorithm. Peter Shor. Prime Factorization. Quantum Logic Gates. Linear Optical Quantum Computing. Boson Sampling. Quantum Computational Advantage.
1980 – Paul Benioff describes possibility of quantum computer
1981 – Feynman simulating physics with computers
1985 – Deutsch quantum Turing machine [18]
1987 – Quantum properties of beam splitters
1992 – Deutsch Josza algorithm is exponential faster than classical
1993 – Quantum teleportation described
1994 – Shor factoring algorithm [19]
1994 – First quantum computing conference
1995 – Shor error correction
1995 – Universal gates
1996 – Grover search algorithm
1998 – First demonstration of quantum error correction
1999 – Nakamura and Tsai superconducting qubits
2001 – Superconducting nanowire photon detectors
2001 – Linear optics quantum computing (KLM)
2001 – One-way quantum computer
2003 – All-optical quantum gate in a quantum dot (Li)
2003 – All-optical quantum CNOT gate (O’Brien)
2003 – Decoherence and einselection (Zurek)
2004 – Teleportation across the Danube
2005 – Experimental quantum one-way computing (Walther)
2007 – Teleportation across 114 km (Canary Islands)
2008 – Quantum discord computing
2011 – D-Wave Systems offers commercial quantum computer
2011 – Aaronson boson sampling
2012 – 1QB Information Technnologies, first quantum software company
2013 – Experimental demonstrations of boson sampling
2014 – Teleportation on a chip
2015 – Universal linear optical quantum computing (Carolan)
2017 – Teleportation to a satellite
2019 – Generation of a 2D cluster state (Larsen)
2019 – Quantum supremacy [20]
2020 – Quantum optical advantage [21]
2021 – Programmable quantum photonic chip
References:
[1] Annalen Der Physik 4(3): 553-563.
[2] Annalen Der Physik 17(6): 132-148.
[3] Physikalische Zeitschrift 10: 185-193.
[4] Proc. Cam. Phil. Soc. Math. Phys. Sci. 15 , 114 (1909)
[5] Physical Review. 21 (5): 483–502.
[6] Proceedings of the Royal Society of London Series a-Containing Papers of a Mathematical and Physical Character 114(767): 243-265.
[7] D. Bohm, “A suggested interpretation of the quantum theory in terms of hidden variables .1,” Physical Review, vol. 85, no. 2, pp. 166-179, (1952)
[8] Physics 1 , 195 (1964); Rev. Mod. Phys. 38 , 447 (1966)
[9] Phys. Rev. 159 , 1084 (1967)
[10] B. R. Mollow, R. J. Glauber: Phys. Rev. 160, 1097 (1967); 162, 1256 (1967)
[11] J. F. Clauser, M. A. Horne, A. Shimony, and R. A. Holt, ” Proposed experiment to test local hidden-variable theories,” Physical Review Letters, vol. 23, no. 15, pp. 880-&, (1969)
[12] (1977) Phys. Rev. Lett. 39, 691-5
[13] A. Aspect, P. Grangier, G. Roger: Phys. Rev. Lett. 49 , 91 (1982). A. Aspect, J. Dalibard, G. Roger: Phys. Rev. Lett. 49 , 1804 (1982)
[14] Europhys. Lett. 1 , 173 (1986)
[15] R. Ghosh and L. Mandel, “Observation of nonclassical effects in the interference of 2 photons,” Physical Review Letters, vol. 59, no. 17, pp. 1903-1905, Oct (1987)
[16] C. K. Hong, Z. Y. Ou, and L. Mandel, “Measurement of subpicosecond time intervals between 2 photons by interference,” Physical Review Letters, vol. 59, no. 18, pp. 2044-2046, Nov (1987)
[17] Phys. Rev. Lett 59, 2153 (1987)
[18] D. Deutsch, “QUANTUM-THEORY, THE CHURCH-TURING PRINCIPLE AND THE UNIVERSAL QUANTUM COMPUTER,” Proceedings of the Royal Society of London Series a-Mathematical Physical and Engineering Sciences, vol. 400, no. 1818, pp. 97-117, (1985)
[19] P. W. Shor, “ALGORITHMS FOR QUANTUM COMPUTATION – DISCRETE LOGARITHMS AND FACTORING,” in 35th Annual Symposium on Foundations of Computer Science, Proceedings, S. Goldwasser Ed., (Annual Symposium on Foundations of Computer Science, 1994, pp. 124-134.
[20] F. Arute et al., “Quantum supremacy using a programmable superconducting processor,” Nature, vol. 574, no. 7779, pp. 505-+, Oct 24 (2019)
[21] H.-S. Zhong et al., “Quantum computational advantage using photons,” Science, vol. 370, no. 6523, p. 1460, (2020)